Years before I considered studying physics, I met Prof. Peter Saulson. The afternoon we met, I was in town to play a concert in Syracuse, New York. The Saulsons helped make the concert possible by providing accommodations at their house for the night.
I shook Peter’s hand at the door and asked, “What do you do?”
He said, “I’m a physicist.”
It’s rare to meet someone so excited about their work that the mention of it makes them grin with gratitude. If they have any social awareness, they have learned not to talk about it too much. I am one of those people. We are like vampires. You must invite us in. If you do, be prepared. You may become one of us.
Maybe that is what happened to me. I asked Peter, “What are you working on?”
Over coffee, he patiently tested how much I knew, and how much I wanted to know, always graciously offering to wrap up the conversation. I could have talked about it for a week. In the years between, we probably have.
While I didn’t learn much about physics in Peter’s kitchen, I learned what it was like to be a physicist. Peter Saulson, a Harvard and Princeton alum and a professor and researcher at Syracuse University, had worked on a single experiment for much of his career — an experiment that had cost over a billion dollars — and it had never shown results. Despite that, he was still excited about it.
The result of the experiment — conducted by a team of a thousand world-renowned physicists, post-docs, undergrads, and engineers — is now well-known. But years ago, in the Saulsons’ kitchen, it was anyone’s guess. I have since come to understand why Peter was not dissuaded, and was perhaps even encouraged, by the conspicuous lack of an outcome.
Peter is now retired. This summer, I called him up and interviewed him for a class assignment. When I asked him why he chose such difficult research he said, “You can fail at anything. Are you working on something for which failure will produce an interesting result?”
A failure to find what they were looking for could have been one of the most intriguing results in the history of physics.
In the 1800s, experimental physicists were able to show that light was a wave. That was exciting because even the most ancient nerds knew how waves worked. Sound waves travel through the air. Water waves travel across the surface of the ocean. Victorian-era physicists hypothesized that light waves must also have a medium, and they called it the luminiferous ether — because back then they could really name things.
Around this time, a physicist named Albert Michelson became known for measuring the speed of light with unprecedented accuracy and designing clever optical instruments. Michelson realized he could detect the ether, this hypothesized medium that filled the universe, if he could precisely measure a difference in the speed of light from two perpendicular directions at once.
If you’ve ever swum in a current, you know how much of a difference the direction you choose can make. Based on this simple physical observation, Michelson hypothesized that light should appear to be moving at a different speed depending on whether it was swimming with, against, or across the ether.
The tool Michelson designed to make this measurement was so ingenious, it is still used in physics labs today. In fact, it is called a Michelson interferometer. True to its name, it measures interference — the way that waves interact with each other.
A modern example of wave interference is noise-canceling headphones. They work not by turning down the noise, but by recording the noise and playing it back “flipped.” The new soundwave peaks when the original dips, and dips when the original peaks. The waves add together but, instead of being twice as loud, they cancel each other. The waves interfere.
The Michelson interferometer works on a similar principle. A single beam of light is split and sent down two perpendicular paths of equal length. Mirrors send the light back, and the two beams join each other again. If the paths are the same length, the beam at the end will look like the original. If the paths are different lengths, or if light takes a different amount of time to travel one of the paths, one beam will destructively interfere with the other.
When Michelson first tested the interferometer, horses and pedestrians walking by his lab jostled the instrument enough to overwhelm the tiny result he was looking for. Rather than being frustrating, this was a good indication of the device’s sensitivity. Michelson enlisted the help of another renowned experimentalist, Edward Morley. They improved the interferometer, installing it on a sandstone slab that floated on a pool of mercury to isolate it from tiny vibrations.
Finally, the instrument was accurate enough to measure the hypothesized movement of the ether. They could turn the interferometer on the pool of mercury, facing one beam in the direction of the earth’s travel and the other perpendicular. They could try it in different seasons, as the earth changed its angle of movement through space around its orbit. As Michelson and Morley turned it, the idea was that one beam of light “swam” against the ether’s current, and the other swam across.
No matter what they tried, they could not detect the ether. The speed of light seemed to be the same in all directions. They considered the experiment — which would produce one of the most revolutionary results in modern science1 — a failure. The news quickly made its way through the physics community.
A wave that traveled through nothing was so counterintuitive that physicists of the time could not imagine a theory without the ether. They even suggested that the “ether wind” shortened one path of the interferometer just enough to cancel out the difference in speed. That sounds crazy — but, to be fair, the truth is equally bizarre. Henrik Lorentz worked out equations for this length contraction that would become important for reasons he couldn’t guess. Henri Poincaré refined this into a robust theory of relativity that included all the funky things that Einstein would say about time and distance — but all this correct work was done with an incorrect understanding.
We all know who Albert Einstein is now, but what you may not know is that Einstein took all this work that others had done to defend the ether hypothesis and flipped it on its head. If the ether existed, it would be the one frame of reference by which all other distances and times could be measured. What if there was no special frame of reference in the universe? What if the speed of light was always the same no matter how the observer was moving? This was Einstein’s insight in the Special Theory of Relativity of 1905. By 1915, Einstein had produced The General Theory of Relativity, an astonishingly accurate theory of how space, time, matter, and energy work together to create and move the universe — no ether required. In 1919, Arthur Eddington observed as the sun’s gravity bent the light of a distant star — just as the theory predicted — and overnight, Einstein became the wild-haired icon of genius that we know today.
Michelson and others never quite gave up on the idea of the ether. His inventions and research won him a Nobel Prize, but he considered his most famous experiment a failure.
As I sat in the kitchen with Peter, he carefully and patiently explained his research. “Gravity can also make waves. They’re tiny, but we think that when very energetic things happen in the universe, like black holes colliding, we might be able to detect that.”
“What is waving?” I asked.
“Spacetime.”
“Space itself is compressing and expanding?”
“Yes. Maybe. We think.”
“And you’re trying to take a picture of these waves?”
“Well, it’s more like a sound than an image. The signal we’re looking for is in a range you can hear, if you convert it into acoustic vibrations.”
The fact that I was getting ready to send acoustic vibrations across a concert hall to this man was not lost on me. I wondered how country music compared to the music of colliding black holes.
In the intervening years, I’ve learned a little about gravitational waves, and the machine they built to find them. Relativity predicts them, but even Einstein was unsure of their existence.
In the 1960s, a few physicists began to wonder if there might be some new technology that was sensitive enough to detect them. Devices were built and failed. In the mid-1970s, radio astronomers Russell Hulse and Joe Taylor observed a pair of stars in a binary system that spiraled closer as they orbited, meaning they were losing energy, and that energy had to go somewhere.2
Several researchers, among them future Nobel Prize winner Rainer Weiss, realized there might already be an instrument that could detect gravitational waves, an instrument that was in almost every physics lab in the world. Over the next few decades, they built prototypes and iterations ever more sensitive and, in 1981, Peter Saulson got involved in the most promising build to date. It was called LIGO.
In September 2015, years after I sat at Peter’s kitchen table, the LIGO collaboration made the first ever verified detection of gravitational waves. When I heard the news, I called Peter to congratulate him. He seemed pleased, but no more excited about the project than when I met him, long before anyone knew if LIGO would show results. Maybe, like anyone who loves to solve problems, he was thinking about the next mystery.
Peter had either never described the engineering aspect of the experiment, or I didn’t know enough at the time to visualize it. I looked around and found a news reel online, and they showed the LIGO facility and explained briefly how it worked.
There are two long perpendicular tunnels. They split a beam of light and send it down both tunnels. Mirrors bounce the light back and the two beams join again.
LIGO is an acronym for the Laser Interferometer Gravitational-Wave Observatory. It is a Michelson interferometer.
Séances were popular in those heady Victorian years. If I could reach Albert Michelson beyond the veil, I would tell him all the amazing work his ideas and innovations have produced. I would tell him what Peter told me.
“You can fail at anything. Are you working on something for which failure will produce an interesting result?”
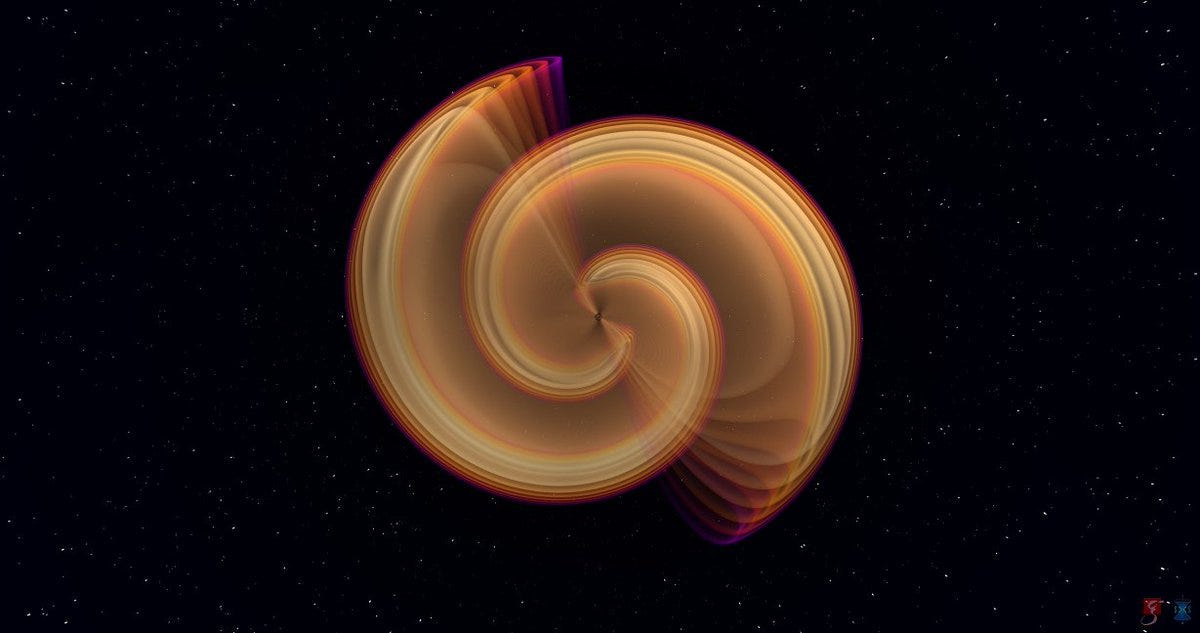
From Peter: “… it might be interesting to say why Michelson considered this “null result” a failure instead of a great discovery. Michelson worried that the ether wind might be impossible to observe in a laboratory on Earth, perhaps because of the building’s roof or perhaps because the atmosphere above the lab somehow entrained the light. In later years, he tried the experiment on a mountain top (Mt. Wilson, I think) If this kind of thing was the problem, then he hadn’t discovered anything but a systematic error in his measurement. He didn’t realize how important this was.”
From Peter: “The rate of energy loss matched what Einstein had predicted for the emission of gravitational waves.”
Special thanks to C. Ellen Honeycutt, Kat Dow, and Peter Saulson.
Share this post